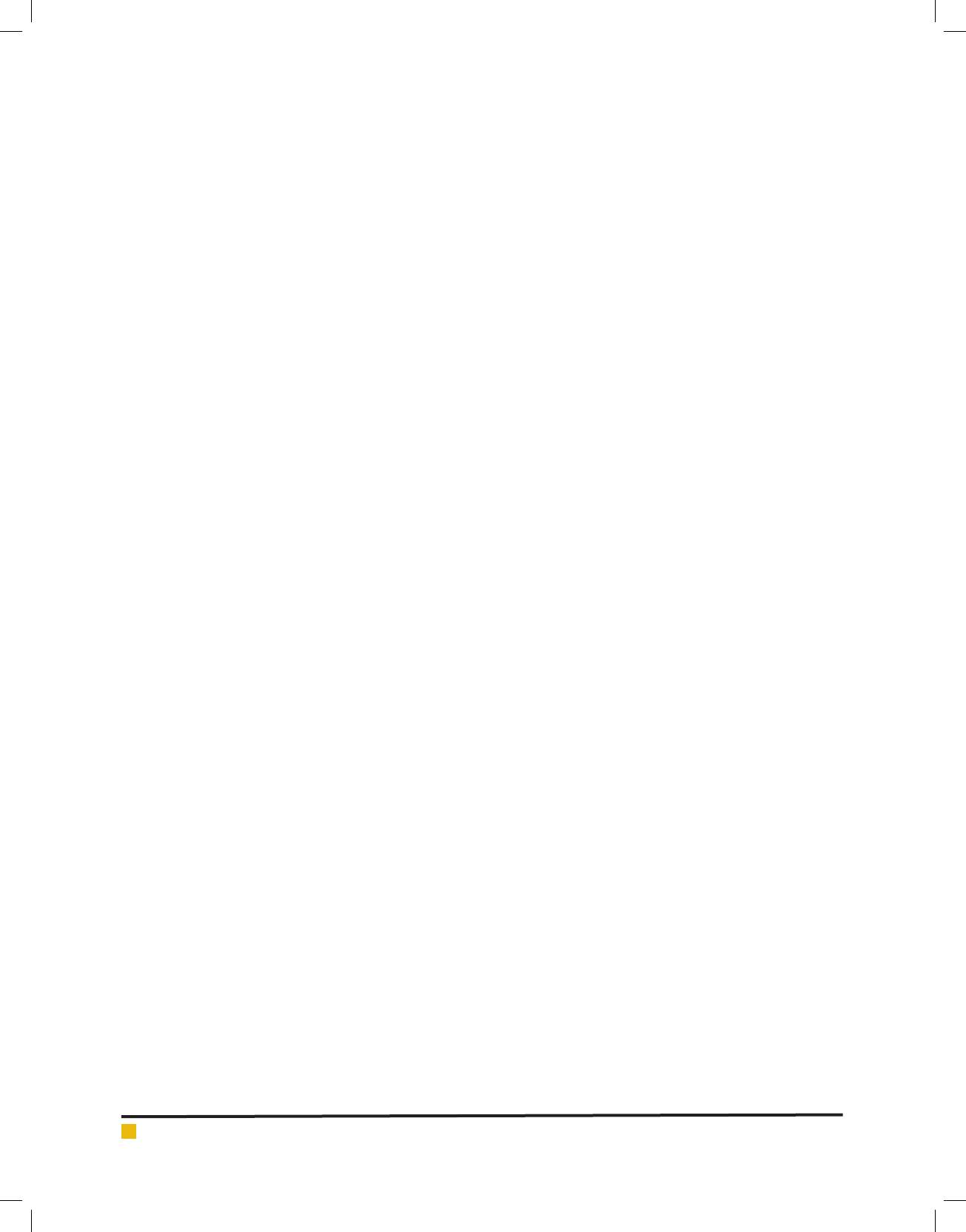
666 UTILIZATION OF DAMAGED AND SPOILED WHEAT GRAINS FOR BIOETHANOL PRODUCTION BIOSCIENCE BIOTECHNOLOGY RESEARCH COMMUNICATIONS
Nadia Razdan and G.S. Kocher
A general acid–base catalyst (McCarter and Withers,
1994; Sinnot, 1990; Tanaka et al 1994) donates hydro-
gen to the glucosidic oxygen and a catalytic base guid-
ing the nucleophilic attack by a water molecule on the
C-1 carbon of the glucose moiety.
Microbial production of glucoamylase
Traditionally, glucoamylase have been produced by
SmF. The development of microbial strains, media com-
position and process control has contributed to the
achievement of high levels of extracellular glucoamyl-
ase. Banakar et al (2012) investigated the amylase pro-
duction from fungal species by submerged fermentation
(SmF). The Production medium was supplemented with
2% (w/v) soluble starch incubated under shake culture at
a temperature of 28±1ºC, pH-7.0 for 7 days. Maximum
amylolytic activity was recorded with crude enzyme at
3rd day of incubation by Penicillium sp. (0.87±0.05 U/
mL) followed by Penicillium chrysogenum (0.69±0.05 U/
mL), Aspergillus candidus (0.67±0.03 U/mL), Aspergillus
fumigatus (0.066±0.06 U/mL) and at 7th day of incuba-
tion was by Penicillium sp. (1.13±0.03 U/mL) followed
by Penicillium chrysogenum (1.12±0.004 U/mL). Wang
et al (2008) investigated food waste (FW) as potential
substrate for the glucoamylase production by Aspergil-
lus niger UV-60 under submerged fermentation. They
reported that optimum concentration of 2.50% (dry
basis), smashed food waste (smashed-FW) produced glu-
coamylase of 126 U/ml after 96 h of incubation, whereas
137 U/ml of glucoamylase could be achieved within the
same time from raw food waste (raw-FW) of 3.75%.
Recently, Okwuenu et al (2017) optimized the produc-
tion of glucoamylase from Aspergillus niger in a sub-
merged fermentation process using amylopectin from
guinea corn starch as the sole carbon source. Speci c
activities for crude enzymes were found to be 729.45 U/
mg and 1046.82 U/mg at ve and twelve days harvested
enzymes, respectively. Benassi et al (2014) investigated
the production of glucoamylase from Aspergillus phoe-
nicis in Machado Benassi (MB) medium using 1% malt-
ose as carbon source. The maximum amylase activity
was recorded with temperature (60–65 °C) and pH (4.5)
after 4 days of incubation in static conditions. However,
the glucoamylase costs are still too high for the estab-
lishment of a cost effective production of energy syrup.
The SSF process has potential to signi cantly reduce
the enzyme production costs because of lower energy
requirements, increased productivity, smaller ef uent
volumes and simpler fermentation equipment (Ellaiah et
al 2002). Cereal bran ours, potato residue and other
starchy waste materials have been utilized as fermen-
tation substrate for glucoamylase production by la-
mentous fungi (Joshi et al 1999; Biesebeke et al 2005).
Glucoamylase production by A. niger was extensively
studied using wheat bran in SmF and SSF by Kaur et al
(2003). Wheat bran, paddy husk, rice processing wastes
or other starch containing wastes have gained impor-
tance as supports for fungal growth during glucoamyl-
ase production (Arasartnam et al 2001).
Sethi and Gupta (2015) isolated amylolytic fungi
from soil and identi ed them as Aspergillus niger, Pen-
cillium chrysogenum, Microsporium sp. and Fusarium
sp on the basis of morphological, biochemical character-
ization and starch hydrolysis assay, of these Pencillium
chrysogenum was most potent alkaline amylase produc-
ing fungi with highest enzyme activity under optimised
conditions i.e pH (8.0), temperature (45°C), wheat bran
(1%) and peptone incubated for 7 days. Indriati et al
(2018) reported that 3, out of 16 thermophile bacteria
produced high amylase activity in media supplemented
with wheat our @ 2% at 40-50ºC.
Zambare (2010) employed response surface method-
ology to optimize SSF medium and various parameters
for production of glucoamylase by Aspergillus oryzae
on the solid surface of rice husk, wheat bran, rice bran,
cotton seed powder, corn steep solids, bagasse powder,
coconut oil cake, and groundnut oil cake as substrates
which resulted in a 24% increase in the glucoamyl-
ase activity. Optimum glucoamylase production (1986
μmoles of glucose/min/g of fermented substrate) was
observed on wheat bran supplemented with 1%, (w/w)
starch, 0.25%, (w/w) urea at pH 6, 100%, (v/w) initial
moisture and 300ºC after incubation of 120 hrs.
Kiran et al (2014) utilized food wastes such as waste
bread, waste cakes, cafeteria waste, fruits, vegetables
and potatoes for glucoamylase production by solid state
fermentation. Response surface methodology was used
to optimize the fermentation conditions for improving
enzyme production and waste cake was the best sub-
strate for glucoamylase production. The highest glucoa-
mylase activity (108.47 U/gds) was achieved at initial pH
(7.9), moisture content (69.6% wt) and inoculum load-
ing 5.2×105 cells/g of substrate and incubation time of
6 days. Kumar and Satyanarayana (2004) improved the
glucoamylase production by a thermophilic mold Ther-
momucor indicae seudaticae in solid-state fermentation
(SSF) by applying response surface methodology (RSM).
The glucoamylase production containing wheat bran as
substrate, under the conditions optimized by RSM, was
455 ± 23 U/g of dry moldy bran (DMB) is higher than
those reported in the literature.
Similarly, Banerjee and Ghosh (2017) applied response
surface methodology, a statistical tool for the optimiza-
tion of glucoamylase production by Aspergillus niger
in solid state fermentation using garden pea peel as a
substrate. The optimized fermentation composition was
incubation time: 5 days; incubation temperature: 30°C;
and substrate amount: 3g, which resulted in GA produc-